High performance polyphosphazene mixed matrix membranes for gas separations
Polyphosphazenes-based membranes have shown considerable promise for post-combustion CO2 capture mainly because of their excellent CO2/N2 gas separation properties. Polyphosphazenes are inorganic-organic hybrid polymers with an inorganic backbone of alternating phosphorus and nitrogen atoms, while the organic groups are usually the two side groups attached to each phosphorus atom. The polymers are synthesized via macromolecular substitution reactions generally by the replacement of chlorine atoms in poly(dichlorophosphazene) by various nucleophiles such as alkoxides, aryloxides, and primary or secondary amines.
Figure 1: Polyphosphazenes offer many unique advantages over traditional polymers.
The phosphorus-nitrogen bonds in the polymers are extremely flexible. Mainly due to the low torsional barrier within the backbone thus making them suitable for high performance elastomers. Other uses include fire retardant materials, electrolytes, optical polymers, and biomedical materials.
If the macromolecular substitution is carried out with polar substituents the resulting polymer has an extremely high affinity for CO2. The base materials selected in this work had both selectivity and permeability. The polyphosphazene base material chosen for this study had a CO2 selectivity of 50(CO2/N2) and a permeability of 500 Barrer. To overcome the needed mechanical properties, we converted the polyphosphazenes into crosslinked interpenetrated networks resulting in significantly improved mechanical properties to cast thin films and with excellent selectivity of 46 (CO2/N2) and permeability of 500. The overall approach for the development of tougher films is outlined in figure 2.
Figure 2: Top) synthesis procedure of interpenetrating network using UV-crosslinking. Bottom) SEM image showing the resulting thin polyphosphazene dense layer on top of the porous support.
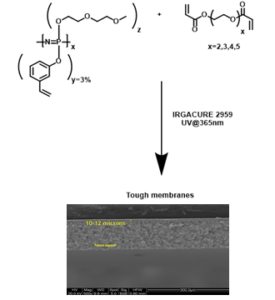
However, it should be noted that within polymeric membrane materials, there is a tradeoff between improving selectivity and permeability. This tradeoff manifests itself in the Robeson upper bound, which establishes upper-limit combinations of permeability and selectivity for the best-performing membranes. And within a polymer class, an increase in permeability is almost always met with a decrease in selectivity. Conversely, some classes of inorganic membranes have selectivities that are many times higher than traditional polymeric materials, but less straightforward fabrication makes these membranes economically infeasible for large-scale applications with ceramic, glass, and zeolitic membranes, sometimes costing several orders of magnitude more than comparable polymer membranes.
One way to enhance gas transport properties and fabricate economically viable membranes which exceed the Robeson upper bound involves forming composite membranes from polymeric materials and inorganic filler particles, yielding mixed matrix membranes (MMM). In theory, the advantages of both the polymer (ease of processing, low cost) and the inorganic material (favorable separation properties) can be realized in MMMs. MMMs have traditionally employed rigid hydrophilic zeolites or carbon molecular sieve particles as the inorganic filler phase, which are usually incompatible with polymers. This incompatibility of surfaces results in a gap (also known as an envelope) between the surfaces of the polymer and inorganic filler particles (Figure 3). Since the gap tends to be much larger than the pores of the inorganic particle, unselective diffusion in the gap dominates selective transport through the particle, failing to realize the performance potential of the membrane. Many other problems may also arise at the polymer/inorganic interface.
Figure 3: Gas transport in mixed-matrix membranes (MMMs) with good material compatibility (left) and poor material compatibility (right).
Most previous research assumed that to surpass the Robeson upper bound, the structure of the MMM had to eliminate the gap at the polymer/filler interface. This “ideal” morphology is impossible due to poor polymer/filler adhesion. We have previously performed work on metal-organic framework (MOF) based MMMs, which were focused on improving the compatibility between the MOF and polymer to eliminate the interfacial gap.
We, along with many other researchers, believed that MOFs’ organic/inorganic hybrid structure should make them intrinsically more compatible with polymers and lend them great promise as fillers compared to inorganic materials. Even making use of these unique materials; however, it has yet proven impossible to fully eliminate the particle envelopment effect and realize the separation potential of the MOFs. The addition of CO2 selective particles does improve the permeability and selectivity, but these improvements tend to be quite small than what is theoretically possible.
During our work on the compatibility of polymers and MOFs, a great deal of understanding was gained on how CO2 diffuses through composite membrane materials. This understanding has led us to conclude that the “ideal” MMM morphology may not be the most desirable for the selective separation of CO2 from N2. We believe that a better solution is to carefully control and take advantage of the gap between the polymer and filler particle rather than trying to eliminate it completely. The interfacial interactions between the polymer and particle will be precisely controlled to accomplish the morphology necessary to create the desired transport. We have coined the term as Interfacially-Controlled Envelope (ICE) membranes to describe the resulting specialized MMMs.
Our approach utilized inexpensive scalable silica nanoparticles commercially available from Nissan Chemical and Evonik corporation (10-15 nm) followed by a simple surface modification reaction to yield material that can allow us to control the interface and thus provide us with excellent membrane properties. We evaluated a few functional groups on the silica particles but found that nanoparticles modified by the cyclohexyl group worked the best in our case. We also found that 40% (TGA) surface modification was optimal. The general synthesis route to make surface-modified silica nanoparticles is outlined in figure 4.
Figure 4: General synthesis for the surface modification of silica nanoparticles.
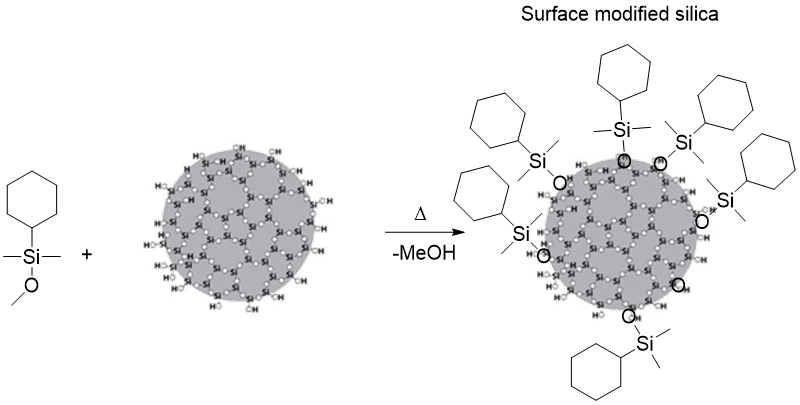
A loading of 30-40% surface-modified nanoparticles was found to be optimal, yielding a selectivity of 44 and permeability of 1600 barriers which is significant as it meant a 3X improvement in permeability without the loss in selectivity.
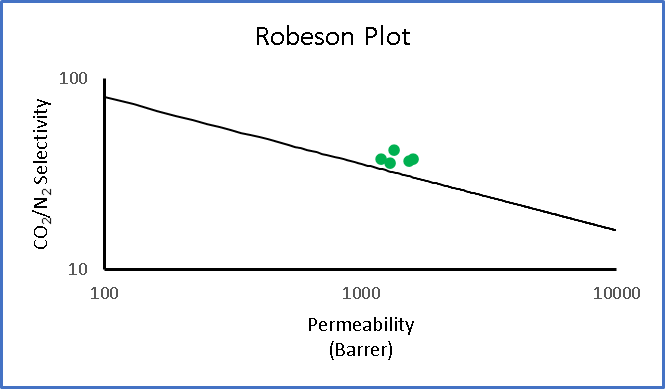
Figure 5: Membrane results for the ICE membrane; note that the ICE membrane supposed the upper bound limits.
In short, polyphosphazenes are excellent materials for gas separation membranes when coupled with surface-modified filler particles. We saw excellent improvement in permeability without the loss of selectivity (selectivity of 44 and permeability of 1600 barriers). The crosslinked interpenetrating approach is a highly useful methodology to improve the toughness of these materials. This work was funded by US-Department of Energy ‘s National Energy Technology Laboratory under the award number DE-FE0026464. A full report on this work can be accessed online at https://www.osti.gov/servlets/purl/1484714.
The work presented here is a joint effort between Prof. Harry Allcock’s Laboratory @ Penn State and RoCo global.
Are you looking for an ideal partner to help you rapidly advance your carbon capture initiatives? Perhaps you want to design and test custom gas separation membranes for your application. Contact RoCo Global today to learn more about our Research & Development Services and how we can help you meet, and exceed, your goals.